WIMI Hologram Academy: The Study of Holographic Communication of Dark Matter Particles
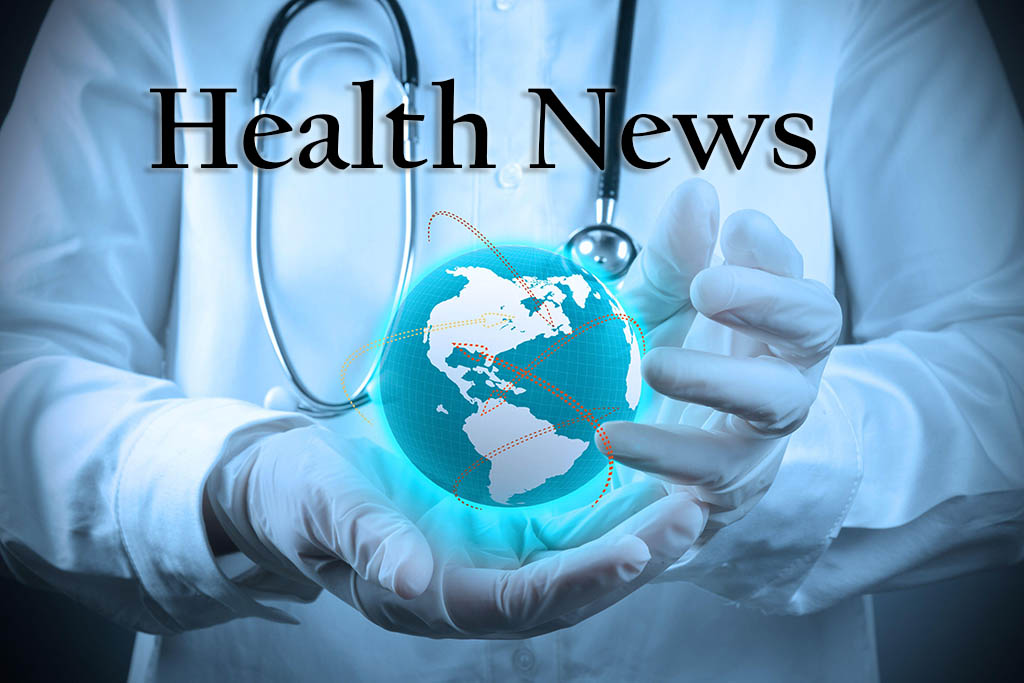
HONG KONG, July 12, 2022 (GLOBE NEWSWIRE) — WIMI Hologram Academy, working in partnership with the Holographic Science Innovation Center, has written a new technical article describing their exploration of Study of holographic communication of Dark Matter particles. This article follows below:
Astronomical observations show that dark matter is widespread in the universe, at five times the abundance of ordinary matter, and that it is accounting for about a quarter of the total energy share of the universe. After nearly a century of exploration, astronomers have discovered dark matter through gravitational observations in the 1930s. Another century puzzle is the origin, acceleration, and propagation of high-energy cosmic rays.The nature of dark matter and the origin of cosmic rays are among the 11 major scientific problems of cosmic physics in the 21st century. The detection of dark matter particles is also a hot spot in countries around the world. The main scientific objective of the dark matter particle detection satellite launched in China is to detect the dark matter particles indirectly through the accurate observation of high-energy cosmic-ray electrons and gamma-rays. As a high-energy particle detector, dark matter particle detection satellite observation data can also be used for cosmic ray physics and related astrophysical studies. Scientists from WIMI Hologram Academy of WIMI Hologram Cloud Inc.(NASDAQ: WIMI), discussed the study of dark matter particles in holographic communication. Based on the data of the dark matter particle detection satellite, the most accurate measurement of the cosmic-ray electron and proton energy spectra is obtained, revealing new structures on the energy spectrum, providing important data for limiting the dark matter particle properties and understanding the origin of cosmic rays. The satellite has also detected about 250 gamma-ray point sources and diffuse gamma-ray radiation from the Milky Way.
Standard cosmological models developed by a large number of astronomical and cosmological observations believe that the energy density of familiar ordinary matter accounts for only about 5% of the total energy density of the current universe today, while dark matter and dark energy account for about 25% and 70%, respectively. Dark matter and dark energy are widely regarded as the new “two dark clouds” facing physics in the 21st century, and the understanding of their nature will surely open the door to a new physical world. The evidence revealed solely by gravitational effects does not know exactly the nature of dark matter, such as whether they are some new elementary particles and how the interaction properties are. To confirm the existence of dark matter particles and to understand their properties, we need to detect other interaction effects beyond their gravity.
Theoretical dark matter candidates vary, ranging from very heavy celestial objects to very light elementary particles, with very large spans of masses and interaction strengths. Among these various theoretical conjectures, a class of candidates called ” weakly interacting massive particles (WIMP) is considered the most likely to constitute dark matter. The patterns of cosmic structure evolution constructed based on such models also coincide with astronomical observations.
AntiMatter particles in cosmic rays are preferred for detecting dark matter particles because an equal amount of positive and negative particles are usually produced if the dark matter particles annihilate or decay. However, the number of positive particles in cosmic rays is far higher than the corresponding number of antiparticles, such as electrons that are about an order of magnitude higher than the positron flow and protons, making it easier to see dark matter left signals in antimatter particles. However, the detection of antimatter particles, especially the high-energy segment antiparticles, can only be based on magnetic spectrometer experiments, which are technically difficult and expensive. Patrons account for about 10% of positive and negative electrons, and dark matter can also be effectively measured very accurately in their total energy spectra without distinguishing between them. The cost of this path will be greatly reduced compared to magnetic spectrometer experiments. This is the concept of the dark Matter Particle Detection Satellite (DAMPE) design. With the support of the Strategic Pilot Science and Technology Special Project of the Chinese Academy of Sciences, the DAMPE satellite was developed in 2015 and successfully launched on December 17 of that year. It has been running smoothly for more than five years and has obtained a large amount of scientific data.
DAMPE has three major scientific goals: through the high precision observation of electron-positron and gamma-ray energy spectrum, direction distribution to indirectly detect dark matter particles, through accurate measurement of wide energy segment nuclide cosmic ray spectrum, and anisotropy study the origin and acceleration of cosmic rays, by observing the gamma-ray research black holes, neutron stars and other extreme high-energy phenomena. To successfully achieve the scientific goal, we identify the high technical indicators that the detector needs to achieve, including the relatively large detection area, 106 x cell detector dynamic range, 104 times electron-proton discrimination, and ~1.5% electron gamma energy resolution.
The DAMPE detector consists of four sub-detectors, which are a plastic flash array detector that detects the particle charge, a silicon array detector that measures the particle direction, and a bismuth germanate energizer that measures energy and performs particle identification, and a neutron detector that assists in particle identification. The plastic flash array detectors measure the incident particle charge by ionization energy damage effects and also act as anti-coincidence detectors for gamma photons. The measured data show that the plastic flash detector can measure the particle charge from Z=1 to Z> 28. The silicon array detector also measures the particle direction based on the ionization energy loss, while also measuring the charge of low-charge nuclides. The direction of the gamma-ray measurement requires first converting it into a pair of positive and negative electrons and then measuring the signal left by the positive and negative electrons passing through the silicon detector. To improve the gamma-ray conversion rate, 1 mm thick tungsten plates were inserted in each of the layers 2,3, and 4th of the silicon microstrip detector. The BGO volonomer is made of 14 layers of bismuth germanate crystal vertically staggered, with a thickness of 32 radiation lengths. For positive and negative electrons and photons, it can detect complete showers, so it is also called full absorption electromagnetic volamer. The BGO energizer has a high energy resolution, and the beam experiment shows that the energy resolution is better than 1.5% above 10 GeV. The BGO energizer can also perform efficient electron and proton discrimination based on the differences in the shower morphology in the energetics generated by the electromagnetic and strong interaction processes. The neutron detector is composed of boron-mixed plastic scintillators designed to assist in distinguishing between electrons and protons. The higher the energy segment, the more obvious the improvement effect of the neutron detector is, so it is crucial for the electronic observation of the energy region above the TeV.
There are two key elements of positive and negative electron observation: one is the accurate measurement of energy and the accurate identification of electron-protons. Correct measurement of the energy requires precise calibration of the detector. On the ground, we can do the energy scale through the accelerator beam, but the high-energy particle detectors operating in orbit have no standard source. We take the minimum ionization particle case for the energizer energy scale. Some cosmic ray cases do not produce showers when they pass through the detector. They deposit energy in the energy gauge mainly through the ionization energy loss process. The energy loss rate per unit length is proportional to the square of the particle charge. When a charged particle reaches the relativistic state, its ionization energy loss rate is only very weakly dependent on the particle energy and thus can therefore be approximated as constant. The simulation calculation can know the energy of this MIP case that should be deposited in the detector. Comparing the electronic reading and the expected physical deposited energy simulated, the energy scale coefficient of the BGO energizer can be obtained. Moreover, the geomagnetic field has a shielding effect on low-energy particles, leading to a truncation of the low-energy spectrum. It can also be tested by measuring the position of the absolute energy mark, which shows that the DAMPE absolute energy mark error is about 2%. The energy target determination is performed at low energy, where the energy standard depends on the linearity of the detector. Each BGO crystal of the DAMPE detector adopts a read-out scheme at both ends. The positive end (P) and the negative end (N) have different gain coefficients, and the measurement results at both ends can be tested against each other. From the data from the beam experiment, the energy measurement from 0.5 to 250 GeV is good. The measured data also show that the energy of the P and N terminal reconstruction remains highly consistent up to about 7 TeV. Through the proportion of measurements on the P and N ends, we obtain a characterization of the energy resolution, which is about 1% for positive and negative electrons in the TeV energy segment, which is consistent with beam experiments and simulation results.
Precise observation of cosmic-ray nuclienergy spectrum in wide energy segments is one of the main scientific objectives of DAMPE. The most abundant ones in DAMPE data are various cosmic-ray nuclei, including protons and helium nuclei. Measuring the nuclide cosmic ray energy spectrum first requires accurate charge measurements. As you can see, both protons and tens of TeV of deposited energy can be clearly distinguished from the charge peak of the helium core, which allows us to screen out very pure proton or helium core samples. Quantitative calculations show that the mutual contamination between protons and helium cores is less than 1% in most energy segments (the deposited energy is less than 10 TeV), and the contamination rate is only about a few percent around 50 TeV.
Unlike positive-negative electrons and gamma-rays, the DAMPE energy can only detect a fraction of the incident cosmic-ray nuclide energy, because strong interactions produce many undetectable secondary particles (such as neutrinos and muons). The fluctuation of the hadronic cascade process also makes the nuclide energy measurement less accurate. Therefore, the transition between the nuclide case energy spectrum and the incident energy spectra recorded directly by the detector cannot be simple. Usually, a method called the energy spectrum deconvolution (spectral deconvolution or unfolding) is adopted to convert the “deposition energy spectrum” measured by the detector into the primary energy spectrum. The energy response matrix of the detector can be obtained by Monte Carlo simulations. After deconvolution and then divided by the effective reception degree of the detector, the primary energy spectrum of the cosmic-ray nuclide is obtained.
DAMPE is also a gamma-ray telescope that can indirectly detect dark matter and study a variety of high-energy celestial phenomena through gamma-ray observations. The number of gamma rays is rare, about one in 100,000 protons, and less than 1 / 100 of the number of positive and negative electrons, so it is critical to identify gamma rays with high accuracy. Gamma-ray discrimination is mainly determined by charge measurement (inverse conformity) of PSD detector and electromagnetic particles and hadronic particles of BGO energizer. Combining the physical quantities from the PSD and BGO observations, a preliminary analysis shows that ~ 250 gamma-ray sources are observed in the DAMPE data, including celestial categories like active galactic nuclei, pulsars and pulsars, supernova remnants, and gamma-ray binaries. One of the major advantages of DAMPE is its high energy resolution, being better than 1.5% above 10 GeV. It is therefore particularly suitable for the search of gamma-ray single-energy linear spectral radiation. The gamma-ray spectrum of high energy (> GeV) is considered a golden means of detecting dark matter because no known astrophysical process can produce such gamma-ray spectral radiation.
Founded in August 2020, WIMI Hologram Academy is dedicated to holographic AI vision exploration and researches basic science and innovative technologies, driven by human vision. The Holographic Science Innovation Center, in partnership with WIMI Hologram Academy, is committed to exploring the unknown technology of holographic AI vision, attracting, gathering, and integrating relevant global resources and superior forces, promoting comprehensive innovation with scientific and technological innovation as the core, and carrying out basic science and innovative technology research.
Contacts
Holographic Science Innovation Center
Email: pr@holo-science. com